Battery materials
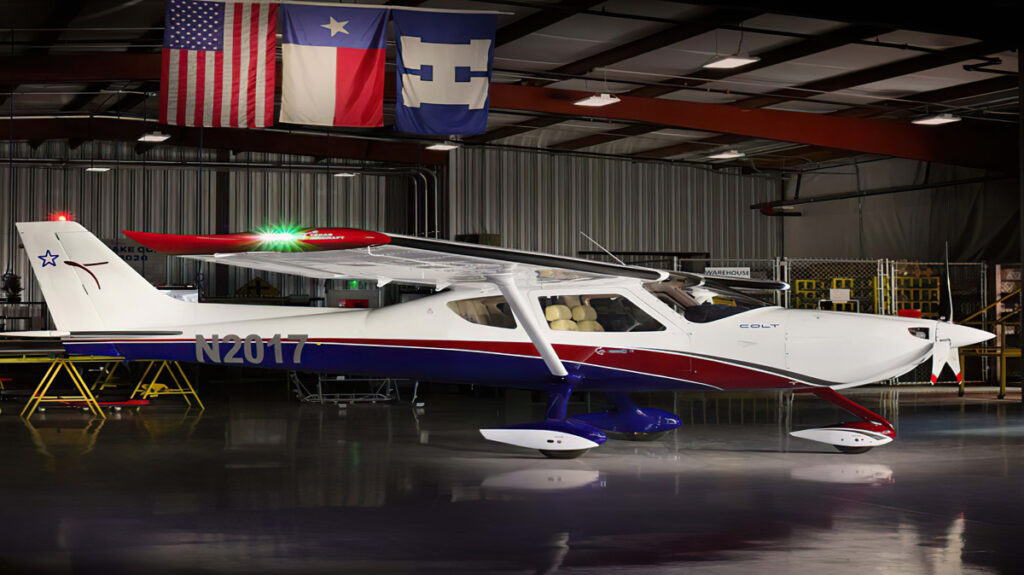
(Courtesy of Oxis Energy)
Nick Flaherty reports on how recent advances in materials are leading to EV batteries with ever-growing energy densities…
Cell growth
Battery systems for e-mobility platforms are based largely around lithium chemistry. Liquid lithium salts with graphite anodes and composite metal cathodes are the dominant combination for battery cells, with variants using nickel, manganese and cobalt or iron phosphate. These have energy densities of up to 250 kWh/kg, but incremental improvements in the electrolytes and battery materials are constantly driving that up. However, some of the alternative materials can be difficult to charge quickly and safely.
At the same time, there have been parallel developments in other materials for the anodes and cathodes to boost performance. Silicon and other forms of carbon, such as graphene and carbon nanotubes, can increase the energy density but have come with a range of challenges. EV makers are now moving to some of these new materials to simplify the manufacturing process to enable cheaper cells.
Battery production is set to grow from 20 GWh a year to 2000 GWh (2 TWh) a year by 2030 and to 30 TWh a year by 2050 to meet the projected demand for EVs. This will come from increasing the number of cells that are built and increasing the energy that each cell produces. All of this requires improvements in the materials used for the cells.
However, there are some fundamental challenges with lithium chemistries. The major issue is the growth of dendrites.
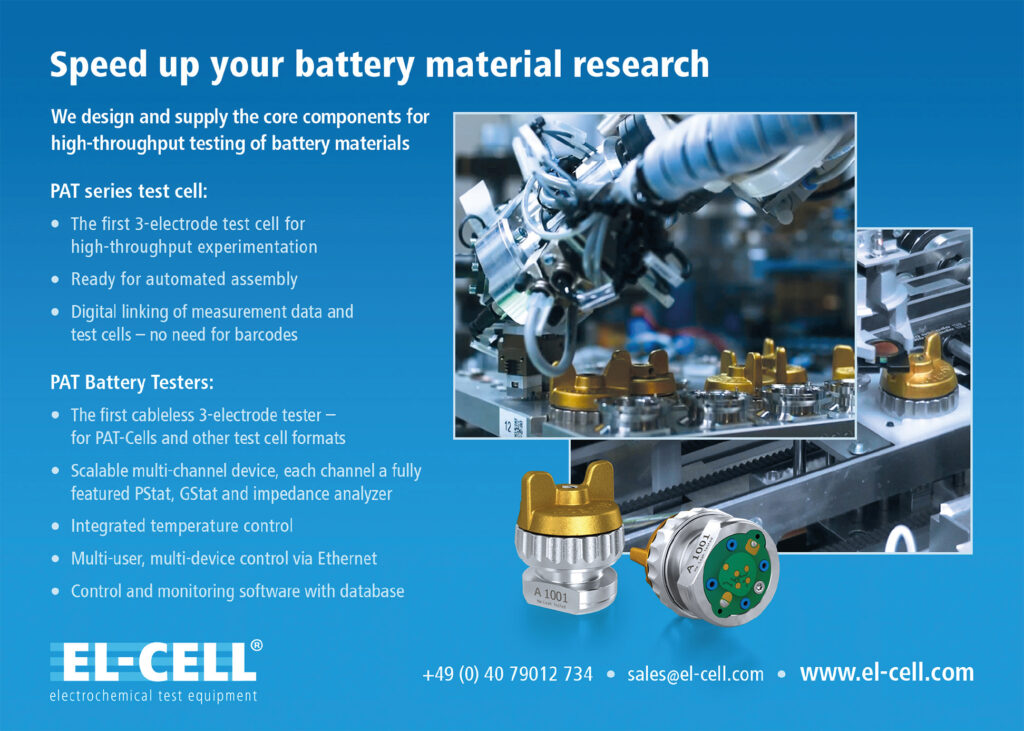
These are tiny, rigid tree-like structures that can grow inside a lithium battery and produce needle-like projections called whiskers. These can pierce the separator layer in the battery, growing until they cause a short-circuit and even cause the cell to overheat and catch fire. This is one reason why the anode cannot be lithium metal, even though it would have a much higher energy density.
Dendrites grow at what is called the solid-electrolyte interphase, a film where the solid lithium surface of the anode meets the liquid electrolyte. A key factor in dendrite growth has been found to be ethylene carbonate, a solvent added to electrolyte ironically to enhance battery performance. Adding more ethylene carbonate speeds up dendrite growth.
This highlights the importance of the electrolyte’s ‘recipe’. It can have multiple constituents, many of which are kept confidential and are a major source of patents. Cell makers are pushing to reduce the number of constituents in the electrolyte but still maintain performance.
The predicted growth in demand for EVs is also spurring the development of completely different chemistries for e-mobility, from lithium-sulphur to lithium-sodium and even aluminium, all of which are cheaper to produce. They have very different energy profiles for battery pack makers and are opening up applications in electric aircraft.
Then there is the move to solidstate batteries, which have solid rather than liquid electrolytes. Having a solid electrolyte creates more of a challenge for the electron migration than the liquid variety but allows faster and safer charging.
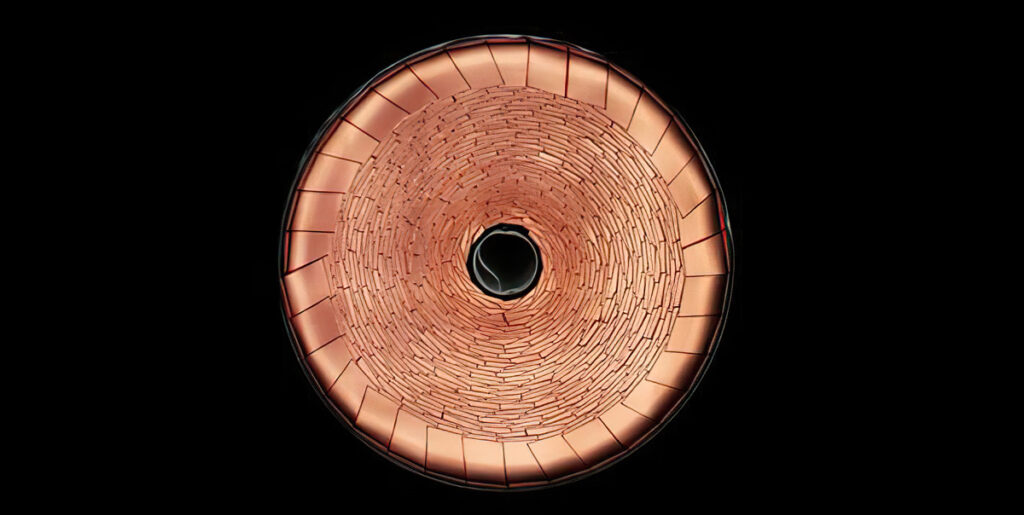
(Courtesy of Tesla)
The materials used in a battery cell are tightly coupled with the manufacturing processes. Many traditional and emerging battery chemistries use pouch cells, which are created in batches and are reasonably easy to build using new materials, although they can be vulnerable to punctures.
Cylindrical cells are harder to make, as they use a rolled-up sandwich of the anode, electrolyte and cathode. But they are more energy-dense than pouch cells, and if steel casings are used they can even be made part of the vehicle’s structure. The choice of materials is key to their cost-effective manufacturing using automated production lines – newer materials may not fit into such lines, or might require complex preprocessing, with nanoparticles for example.
Then there is a move to eliminate the cobalt used in modern nickel manganese cobalt (NMC) lithium-ion cells. It comes from unsustainable sources around the world, and there are challenges with creating a pure nickel cathode that is thin enough for a rollto- roll process but thick enough not to crack in the same process. That roll can be up to 1 m long.
All this is driving tremendous amounts of innovation in existing materials for lithium-ion batteries.
Electrolytes
A new generation of electrolytes is boosting the performance of existing NMC lithium-ion cells. Organosilicon (OS) electrolytes for example can improve the cycling stability of cells that use NMC622 or NMC811 cathode materials. The former has six parts of nickel to two parts of manganese and two parts of cobalt, hence NMC622; the latter has 8, 1 and 1.
These are used in combination with various state-of-the-art anode materials and with silicon graphite composite anodes. That can help raise the energy density from 250 to 400 Wh/kg with silicon anodes and reduce the cell’s overall cost by using higher proportions of nickel in the cathode.
The combination of OS electrolytes and high-nickel NMC cathode materials has been used in early demonstrations to enable high-performance lithium-ion battery cells with lower costs, longer lifetimes and fast charging.
Carbon
The first step for improving lithiumion cell designs has been to look at changing the structure of the carbon used in the anode. For example, the latest roll-to-roll process uses a ‘dry electrode’ process, with the powdered graphite layered on a foil without having to use solvents. How materials fit into this process is just as important as the performance of the material itself.
Carbon nanotubes, using 2D sheets of carbon (graphene) rolled into tubes, have been a strong area of research. These can be used as a composite with a range of lithium-storing materials, such as silicon, graphite and metal oxide particles. This strengthens the composite, allowing high-performance electrodes with thicknesses of up to 800 μm that are thin enough for a roll-toroll process.
These composite electrodes are then used to build cells with an energy density of 480 Wh/kg‑and 1600 Wh/litre – more than twice that of current cells, providing twice the battery life for the same size.
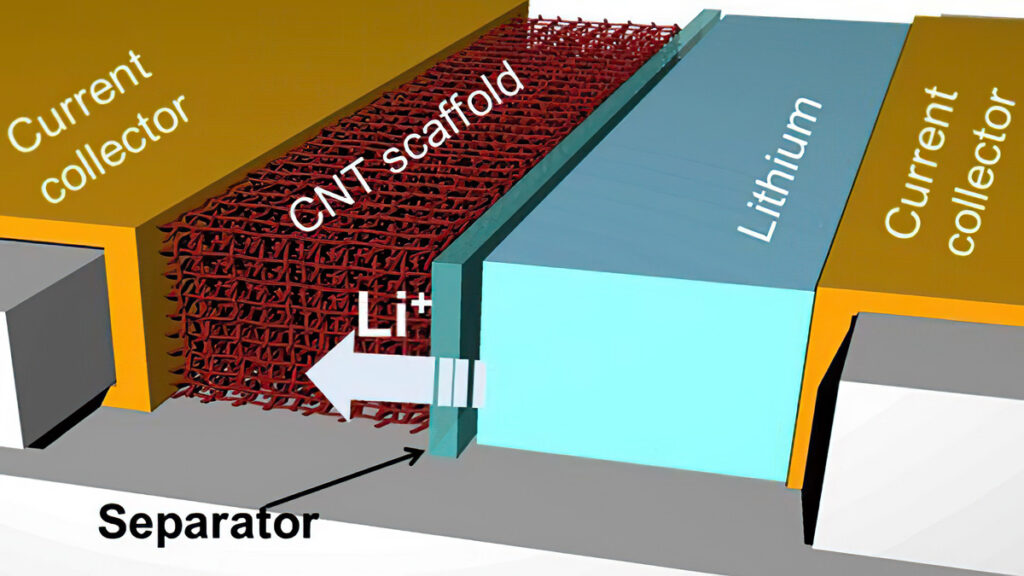
(Courtesy of Juran Noh, Texas A&M University)
Carbon provides areal capacities of up to 45 mAh/cm2‑for anodes and 30 mAh/cm2‑for cathodes, and combining optimised composite anodes and cathodes yields full cells with an areal capacity of 29 mAh/cm2to achieve the breakthrough power density.
One approach, vertically aligned carbon nanotubes (VACNT), provides a 3D-structured electrode rather than one in a disordered powder form. This can improve the energy storage by a factor of three, with five times the cycle life. At the same time, it can cut charging times to minutes.
All of this can be done on a rollto- roll process to maintain the anode structure in a high-speed manufacturing process rather than using an engineered powder such as silicon.
However, the VACNT approach can also be used with conductive polymers as well as lithium, sodium, silicon, sulphur and titanate materials to build electrodes, and works with a range of electrolytes. This is also being developed to provide more structural strength so that the batteries can be part of body panels in vehicles.
Another approach enabling fast charging times coupled with hundreds of thousands of charging cycles will help to reduce the strain on lithium-ion batteries.
The key difference from other carbon materials is a curved graphene carbon material used for ultracapacitors that allows for fast charge-discharge. This is combined with a specifically developed battery electrolyte this can charge in 15 seconds and discharge with high energy efficiency, while still exhibiting a greater energy density than traditional lithium-ion cells.
Silicon
Silicon has also attracted attention for lithium batteries owing to its high specific capacity, which is nearly 10 times that of graphite. However, while it takes more lithium ions to store more energy it swells by a factor of four, which can cause cells to split, leak and even catch fire.
Although silicon is cheap and production is well-established for integrated circuits, it is brittle and doesn’t fit easily into a roll-to-roll process. So there are many start-ups and large organisations looking at how to make it suitable.
There are multiple approaches to using silicon. A silicon-based composite powder can be used in hybrid or lowloading anode electrodes with up to 10% silicon alongside graphite. This can provide an initial anode capacity 400- 450 mAh/g. For higher loads with up to 80% silicon, nanoparticles of silicon are engineered to avoid expansion, and can provide a capacity of 2000 mAh/g but with limited stability.
Another form of silicon being used is a porous 10-60 μm film layered directly on a copper foil without using a binder, and is suitable for a roll-to-roll process. This is expected to be used in electric cars from 2024.
This scalable nanomaterial is made using silicon nanoparticles grown on nanographite flakes by aerogel fabrication. Silicon powder is mixed with the nanographite mixture to give a stable specific capacity, even at high current rates, and good cyclic stability. The specific capacity is 455 mAh/g for 200 cycles.
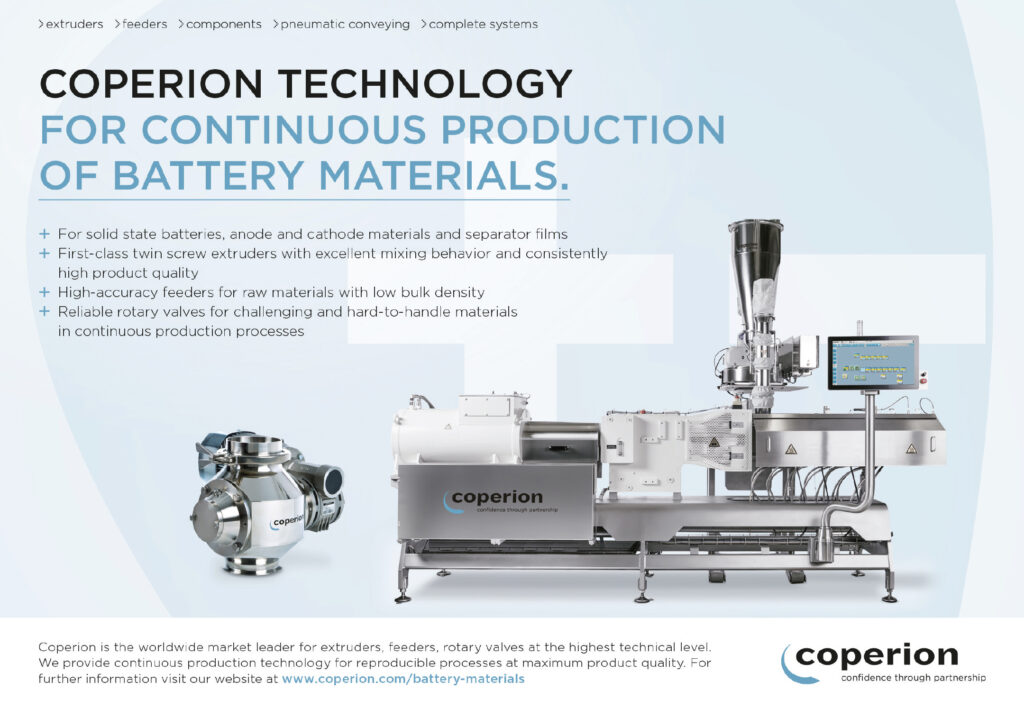
Nanoparticles can be tricky to make, however, which is why there is a search for materials that inherently have the right properties even with comparatively large, micron-sized particles. This avoids the need for a complicated process to make them, which keeps costs low.
Nanoparticles are also challenging to work with on a practical level, as they tend to be quite ‘fluffy’, making it difficult to pack them tightly together, which is key for a battery’s volumetric energy density.
Many start-ups and research labs have produced prototype cells, but the move from the lab to production is a huge step. Rather than using an engineered material, using base silicon with an elastic ion-conducting polymer coating, then a highly elastic binder, costs just $1.2/kWh. This increases the vehicle range available from the cell by 20% while reducing the cost of the whole battery pack by 5%.
Sulphur
Lithium-sulphur (LiS) is an emerging chemistry that provides a high energy density in lightweight cells. It is of growing interest for electric aircraft, large commercial vehicles such as buses and marine applications, where a lighter battery pack can increase the range, and is currently produced in pouch cells.
The combination of lithium and sulphur can provide a theoretical energy density of 2700 Wh/kg, but there is a practical upper limit of 470 kWh/kg, which is twice that of high-energy lithium-ion cells. Sulphur is also cheap, as it is a by-product of the oil industry.
LiS also has good safety characteristics regarding the internals of the cell. Inside the cell is a passivation layer, where the sulphur coats the lithium to allow the cell to stand up to short-circuit tests and even bullet penetration tests. While dendrites form from the lithium, these erode away, forming a ‘mossy’ layer that is part of the cell’s degradation.
In a LiS cell the cathode is primarily sulphur, mixed with carbon to provide conductivity and a binder to hold them together. One of the key drivers for cell designers is to reduce the amount of carbon and maximise the amount of sulphur in the mix.
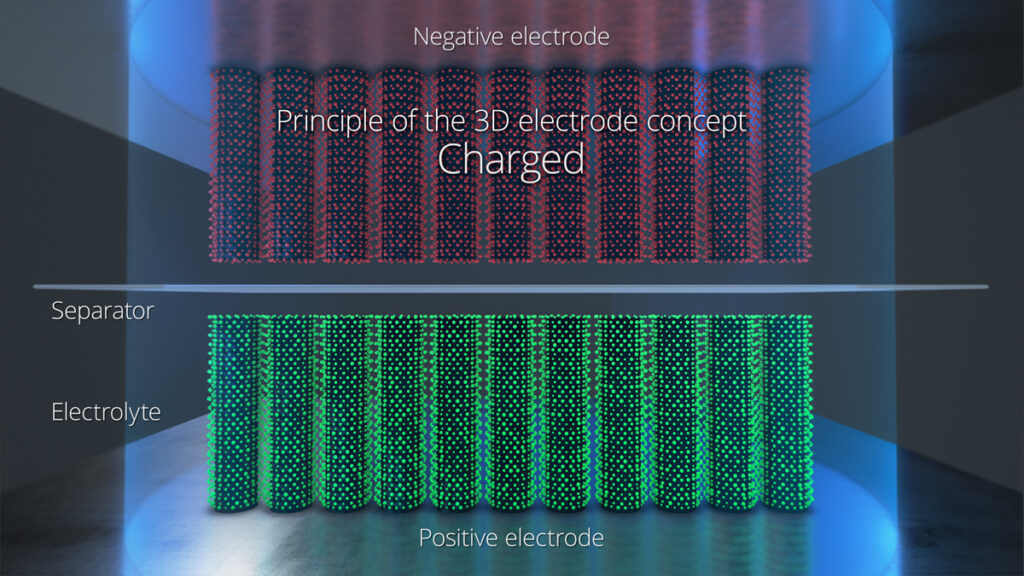
(Courtesy of NAWA Technologies)
The anode is a lithium metal foil, and that is evolving by using thin layers of a ceramic to provide a coating and improve the cell’s lifetime by reducing that mossy dendrite layer. These ceramics must have high ionic conductivity yet be mechanically and chemically robust.
The contacts in LiS pouch cells use aluminium foil, as the size and mass of the contact has to match the cell’s power output. If the contacts are not matched then resistive heating will occur.
LiS pouch cells also use a manufacturing process that is very similar to that for lithium-ion cells, allowing the same equipment to be used and making the process costeffective. Most of the design is in the electrolyte and the cathode.
One of the challenges for LiS cells is the charging rate. It currently takes a few hours to charge a pack, although improvements in the materials will see a pack charging in an hour. Fast charging in a matter of minutes is a challenge, but the light weight of a LiS cell means a larger pack with more energy can last two or three days between charges.
For fast turnaround for aircraft, packs could be exchanged rather than waiting to charge. The technology is now being used for six-seater aircraft.
Sodium
Work on sodium batteries has been ongoing for the past 20 years, but rather than taking on lithium-ion cells directly, the technology is looking at replacing lead-acid batteries. It is finding applications in small packs for electric bikes and three-wheelers, and large 250 kWh packs for trucks.
At 150 Wh/kg for a large, 12 Ah pack, the energy density is comparable to lithium-ion but at lower cost and offering greater safety. Optimising the design of the elements in a cell will produce a 190 Wh/kg cell in 2021 with a capacity of 30 Ah.
This cell uses sodium nickel oxide cathodes, where nickel is about a third of the electrode in the form of a hard, glassy nickel manganese material called spinel.
Like LiS, sodium cells can be built on a lithium-ion production line with minimal modifications, and changing the current collectors to aluminium on both sides of the cell.
The anode is hard carbon, as graphite cannot be used because the sodium ions aren’t easily incorporated, or intercalated, into the graphite to release the energy. Hard carbon is a disordered form of carbon that has a short-range layers structure, and the insertion process for the sodium is different. Some lithium-ion cell makers also use the material, which is made from a biomass such as coconut, and it is available from three or four major suppliers.
Using hard carbon limits the energy density, with a capacity similar to graphite but a different voltage profile, and with more of a plateau in the discharge curve. This has an impact on the algorithms used in the battery management system.
The electrolytes are also different in a sodium battery, using NaPF6 in linear and cyclic fluorinated carbonates rather than LiPF6.
The intellectual property is more around the additives than the basic electrolyte materials, which are similar to lithium-ion. These additives modify the electrode interface and make the interface more stable. That is key to getting better cycle life, currently retaining 80% of the charge in the cell over 3000 cycles, which corresponds to a 10-year battery life.
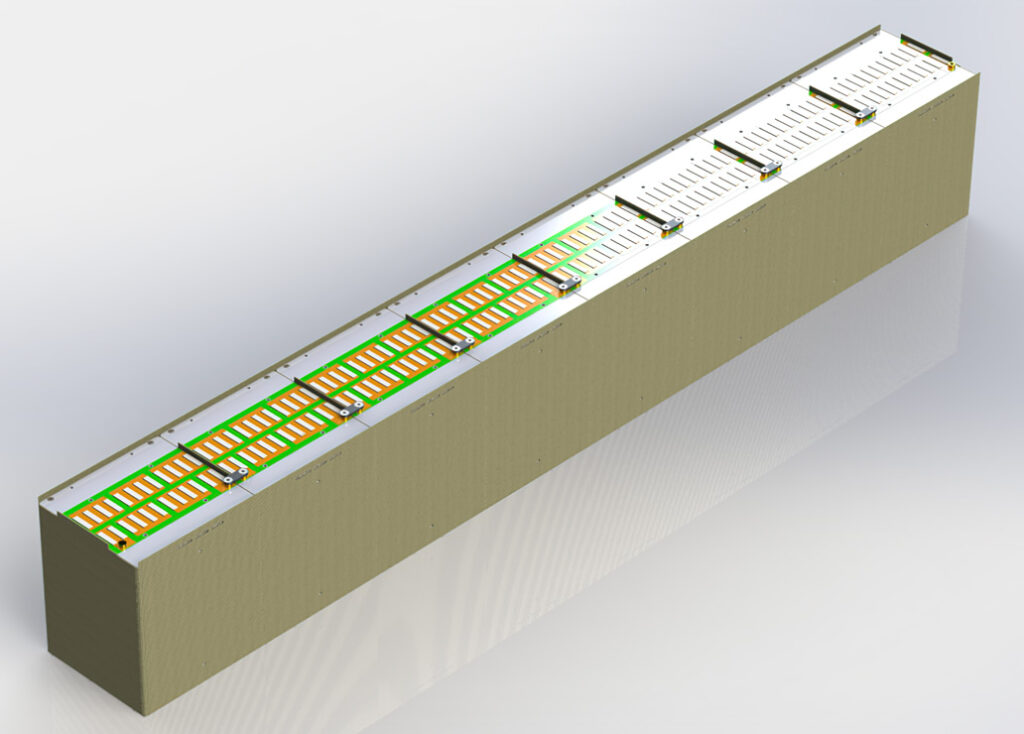
A lithium-sulphur module concept for aerospace applications using a modular design approach, enabling the modules to be distributed equally around the aircraft
(Courtesy of Oxis Energy)
Ethylene carbonate is the weakest link in the electrolyte used for the dielectric constant. A graphite electrode can’t use propylene carbonate, which is unfortunate as it’s cheap and has good dielectric properties, but using hard carbon avoids the need for it. It also freezes at lower temperatures than the electrolyte in lithium-ion cells, down to -30 to -40 C.
A sodium cell can also handle a fastcharging process, up to 6C. That can charge a pack to 80% in 15 minutes. Another advantage of using sodium is that the cells can be stored and transported at 0 V, making them entirely safe for transportation in the air.
Aluminium
The aluminium-air battery is considered to be an attractive option in some quarters, because of its theoretical energy density of 8100 Wh/kg – dramatically higher than lithium-ion. Aluminium is also the most recycled metal on the planet and is economically cheap compared with lithium, zinc and magnesium.
There are systematic challenges as well as challenges with the material though. Creating suitable alloys to reduce the corrosion of the metal is one, as aluminium oxide builds up and reduces the battery’s effectiveness.
In the cathode, extensive studies of electrocatalytic materials for oxygen reduction have used platinum and platinum alloys, non-preciousmetal catalysts andcarbonaceous materialsfor the air cathode. Aqueous andnonaqueous electrolyteshave also been examined, with inhibitors added to the electrolyte to enhance the electrochemical performance.
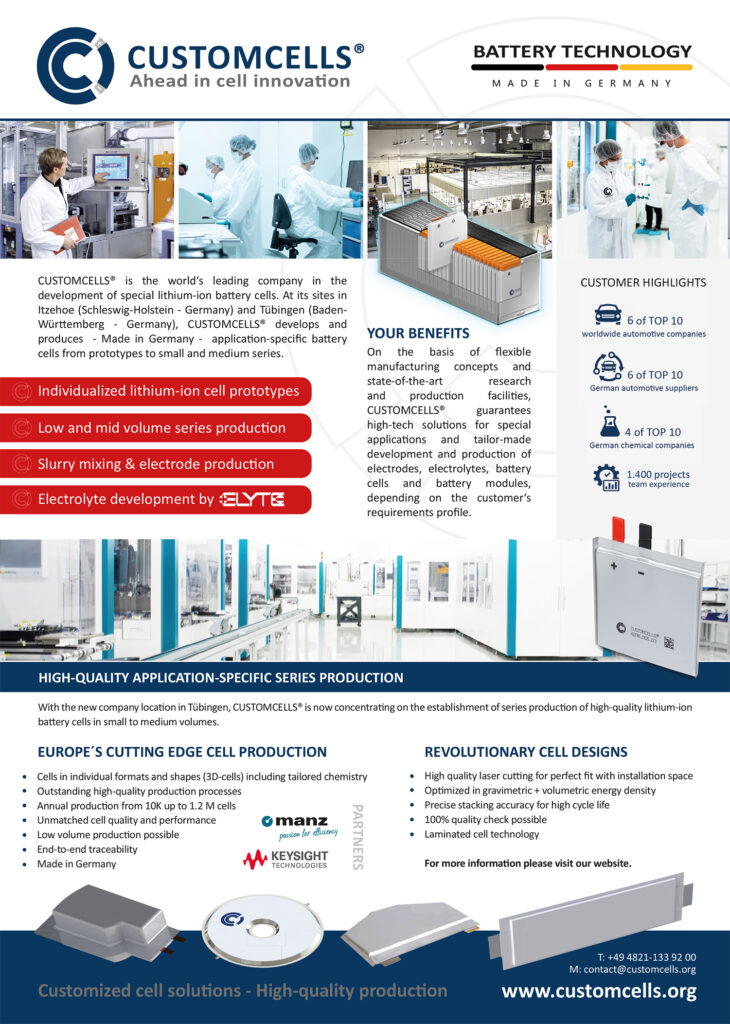
The non-aqueous systems can prevent electrode corrosion, hydrogen evolution and electrolyte drying, unlike aqueous electrolytes. In particular, polymer electrolytes are extremely attractive for aluminium-air batteries thanks to their fl exibility, light weight and increased thermal stability. However, the relatively low ionic conductivity of polymer electrolytes at room temperature is a challenge that is still to be overcome.
Aluminium-air is also a primary, nonrechargeable cell, so early versions had to be replaced rather than recharged. Development of a solid-state version has enabled a rechargeable aluminium-air battery with a solid electrolyte composed of AlCl3, urea and glycerine to be fabricated.
The battery exhibited stable electrochemical reactions, and when TiN was used as an air cathode, the typical byproducts of an aluminium-air battery such as Al(OH)3and Al2O3were not observed, for the fi rst time, even with a solventbased electrolyte. The TiN air cathode surface was covered with a layer that did not hinder the electrochemical reaction.
All this has led to a 100 kg aluminiumair battery pack that has been used to power an EV.
Magnesium
Magnesium is also of increasing interest as a battery material. The European Magnesium Interactive Battery Community research project for example aims to combine all the steps for developing magnesium batteries, from basic research to cell production processes, but has at least two more years to run.
Other developments combine a nanostructured cathode and a new understanding of the magnesium electrolyte. This allows batteries with a capacity of 400 mAh/g, up from 100 mAh/g for earlier magnesium batteries and twice that of commercial lithium-ion batteries, with a cathode capacity of 200 mAh/g.However, the voltage of the new version remains low, at about 1 V, compared with 3-4 V for lithium batteries.
Magnesium ions are hard to insert into an electrode, as it is diffi cult to break the magnesium bonds. More than that, the ions move extremely slowly in the host. All that lowers the battery’s effi ciency.
Increasing the gaps in the cathode from 0.57 to 1.8 nm allows the magnesium ions to be inserted more easily though. This increases the energy capacity with faster charging at room temperature.
There are also battery cathodes based on titanium fl uoride phosphate that use potassium rather than lithium. The KTiPO4F cathode for example has a high electrochemical potential and high levels of stability at high charge-discharge rates.
The potassium-ion cell has an electrode potential of 3.6 V, and a carbon-coated battery cathode showed no reduction in capacity cycled at 5C fast charging for 100 charging cycles.
Solid-state batteries
Solid-state batteries are a key area of development for e-mobility applications, and there is a focus on the electrolyte rather than the electrodes. Using a solid electrolyte rather than a liquid provides more safety and allows larger, lighter batteries. The latest solid-state lithium metal battery technology is reaching 400 Wh/litre, approaching that of the best polymer cells, with 1C charging in 2 hours.
Solid-state materials look set to extend the energy density to 1000 Wh/litre by 2024 forlong-range EVs, with 3C charging of a pack in 20 minutes.
One example, a solidnanocomposite electrolyte, has a conductivity of up to 10 mS/cm, and has the potential to become even higher. It is applied as a liquid via wet-chemical coating, then converted into a solid when it is in place in theelectrodes. That allows it to be cast into densepowder electrodes where it fi lls all cavities and makes maximum contact in the same way as a liquid electrolyte.
Again, this allows the cells to be built using the same equipment as existing technologies.
Another approach for solid-state batteries is to use lithium-conducting polymers combined with protective layers of sol-gel materials – a method for producing solid materials from small molecules. This is a wet-chemical technique used for fabricating glassy as well as ceramic materials. The sol(or solution) gradually forms agel-like network containing both a liquid and a solid.
Fast charging
Researchers in the UK have identified a group of materials that could be used for fast-charging batteries without having to use more expensive nanomaterials.
Niobium tungsten oxides do not result in higher energy densities but allow lithium ions to move much faster through a battery. The physical structure and chemical behaviour are also inherently safer. Similar materials are being used in Toshiba’s fast-charging solid-state batteries, for example.
The oxides used in the current research have a rigid, open structure that does not trap the inserted lithium. They also have larger particle sizes than many other electrode materials.
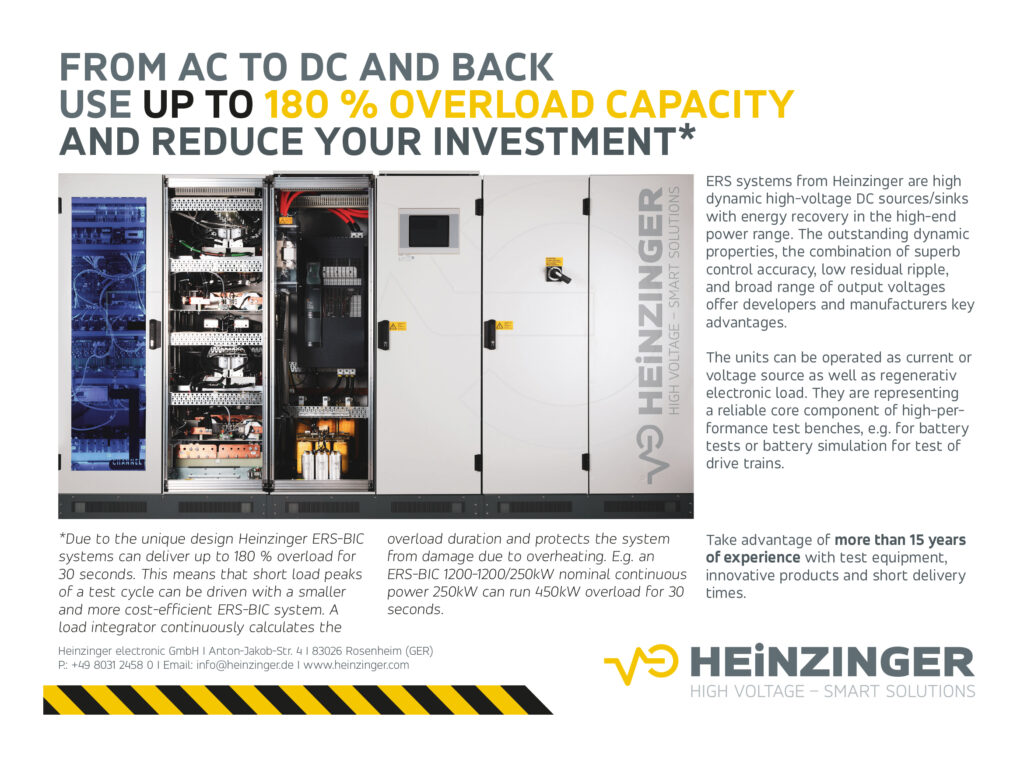
Many battery materials are based on the same two or three crystal structures, but the niobium tungsten oxides are held open by ‘pillars’ of oxygen, which enables lithium ions to move through them in three dimensions. The oxygen pillars, or shear planes, make these materials more rigid than other battery compounds so that, with their open structures, more lithium ions can move through them, and far more quickly.
As well as their high lithium transport rates, the oxides are also simple to make. A lot of the nanoparticle structures take multiple steps to synthesise, and produce only a tiny amount of material, so scalability is a real issue. The oxides however don’t require additional chemicals or solvents.
Although the oxides have excellent lithium transport rates, they do lead to a lower cell voltage than some electrode materials. The researchers say though that the lower operating voltage has safety benefits.
The new anode structure has increased the fast-charging time of a solid-state battery to provide a range of 320 kmwith 6 minutes of fast charging and a lifetime of 5000 cycles.
The SCiB (Super Charge Ion Battery) usesa titanium niobium oxide anode material that has double the lithium storage capacity by volume of the graphite-based anodes generally used in lithium-ion batteries. This compares to theprevious version, which uses a lithium titanium oxide anode but has a lifetime of 20,000 cycles.
The anode is also much less likely to experience the formation of dendrites during rapid charging.
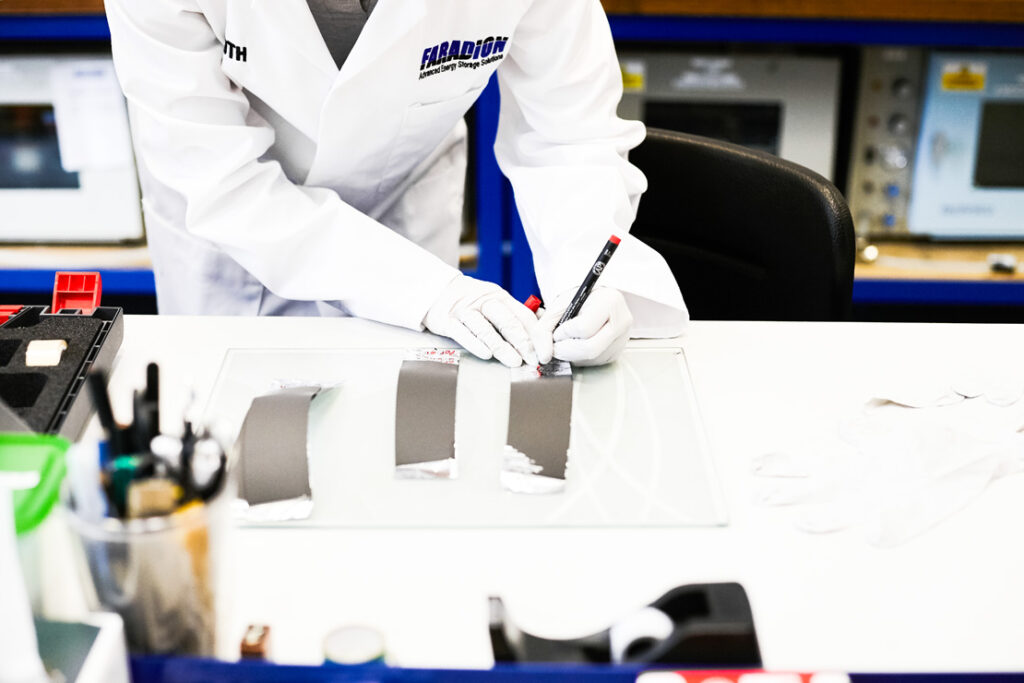
Lithium-sodium pouch cells can be built using the same equipment as lithium-ion cells
(Courtesy of Faradion)
Creating this new cell required a new method for synthesising and disarranging crystals of titanium niobium oxide, and storing lithium ions more efficiently in the crystal structure. The anode of the next-generation SCiB has twice the capacity of the anode of current lithium-ion batteries. The present 20 Ah SCiB cell has an energy density of176 Wh/litre.
A 50 Ah prototype of the new battery technology keeps the long life, low temperature operation, excellent safety and rapid recharging characteristics of the current SCiB but with double theenergy density by volume. The nextgeneration SCiB alsomaintains more than 90% of its initial capacity after being put through 5000 charge-discharge cycles, and ultra-rapid recharging can be done in cold conditions, at temperatures as low as -10 C, in just10 minutes.
The technology has recently been approved for use in marine vessels from Nippon Kaiji Kyokai in ClassNK vessels to provide electric power when entering or leaving port.
Machine learning
There is a hugely complex balance of all kinds of combinations of materials for a cell’s anode, cathode and electrolytes, which is difficult to analyse and model for the best energy density, cycle times and fast charging.
Synthesising ionic compounds and measuring ionic conductivity are labour intensive tasks, and experimental results can be difficult to interpret. Instead, computational methods and machine learning are easy to automate and run in parallel to efficiently identify materials that merit the time and expense of experimental investigation in the search for new electrolytes, particularly for the solid-state varieties.
Current approaches to computational screening of battery materials rely on simulations of the electronic structure to determine the insulating character of a material and on molecular dynamics simulations to predict the lithium-ion diffusion coefficients.
That means running thousands of calculations, so automation and reproducibility are essential, but these computational methods also need to be inexpensive enough to be run for thousands of materials, yet accurate enough to be predictive.
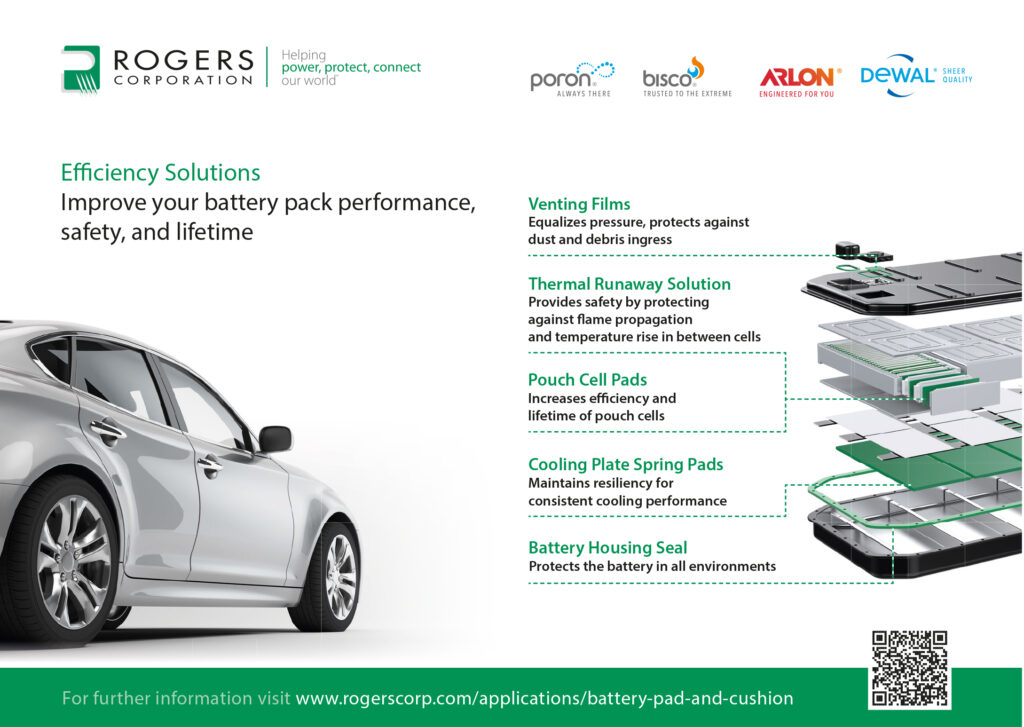
So, machine learning techniques are being used to find new combinations of materials for battery cells. These draw on vast databases of existing materials, along with their manufacturability, to explore new combinations that might yield significantly greater results. This could allow new cell designs to be developed and tested that were not previously considered.
One approach is to use a technique called computational screening to look for new solid-state battery materials.
The results are being made available in an open source tool.
The computational framework is used for predicting the diffusion of lithium ions in solid-state electrolyte materials. The framework shows how large-scale computational screening can identify new ceramic compounds for further investigation.
Used with novel cathode and anode materials, these could prevent the growth of lithium dendrites.
The approach has been applied to screening two repositories of experimental structures that describe around 1400 unique crystal structures. After identifying electronically insulating systems that can’t be used, a technique called a pinball model was used to identify materials likely to display fast ionic diffusion.
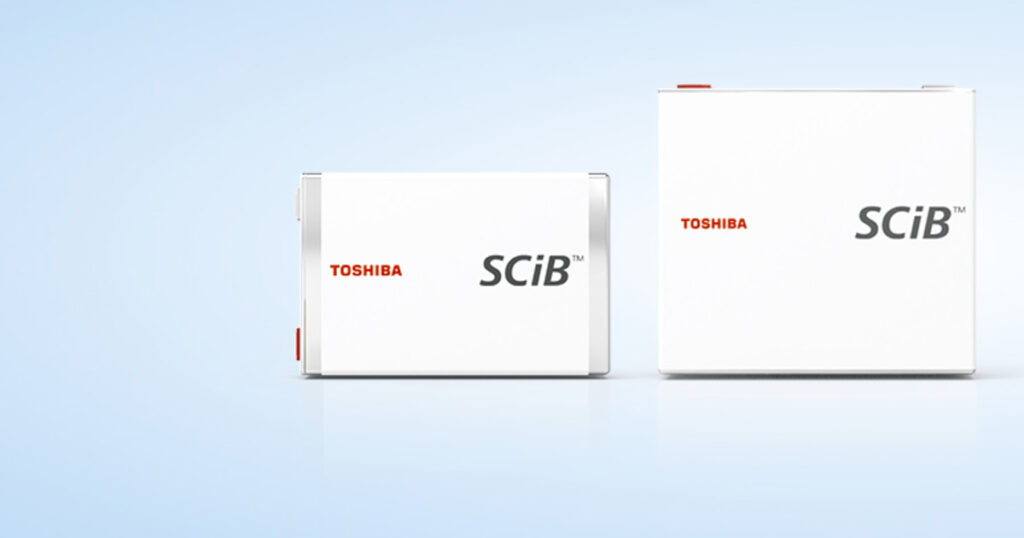
(Courtesy of Toshiba)
The model is a framework based on physical observations of how electrons behave in an ionic system, which simplifies the modelling of ionic conductors. More than 115 identified structures were then simulated with accurate first-principles molecular dynamics for a total of 45 ns at high and intermediate temperatures.
The approach resulted in the identification of five solid-state battery materials with fast ionic diffusion – some in the range of the superionic conductor Li10GeP2S12 – as well as 40 materials that at least showed significant diffusion at 1000 K.
Although it is not possible to say whether these latter materials can be considered fast-ion conductors at lower temperatures, because of the short timescales of the study, they are identified for more detailed work.
Acknowledgements
The author would like to thank Dr Mark Crittenden at Oxis Energy, Jerry Barker at Faradion, Drew Baglino at Tesla and Ulrik Grape and Pascal Boulanger at NAWA Technologies for their help with researching this article.
Some suppliers of battery materials
Australia
Canada
China
France
Germany
Israel
UK
Bacanora Lithium
Faradion
Oxis Energy
Toshiba Europe
+44 (0) 20 7920 3150
+44 (0) 114 224 2421
+44 (0) 1865 407017
+44 (0) 020 8734 5800
USA
Enevate
Panasonic
Paraclete Energy
+1 949 243 0399
+1 877 803 8492
+1 734-288-4120
ONLINE PARTNERS
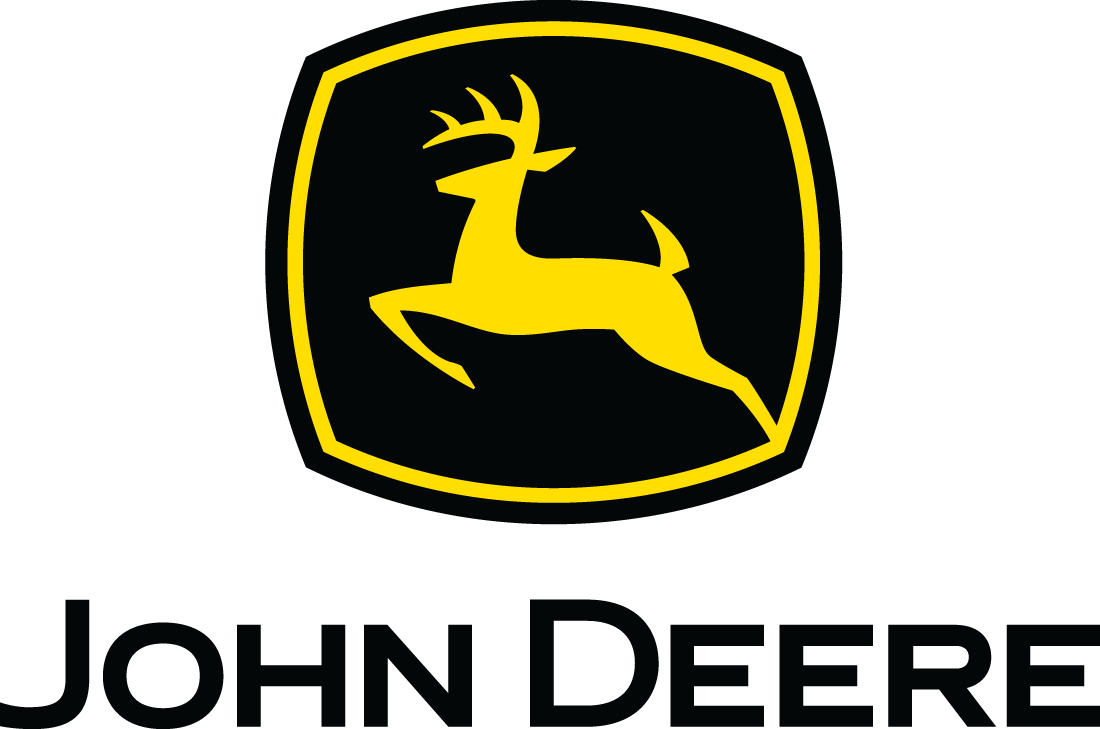
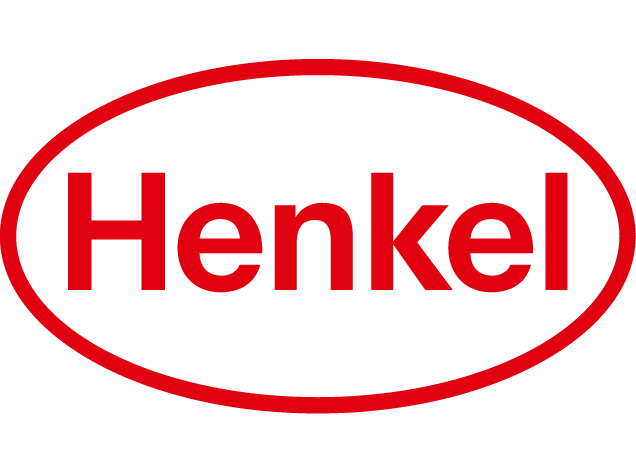
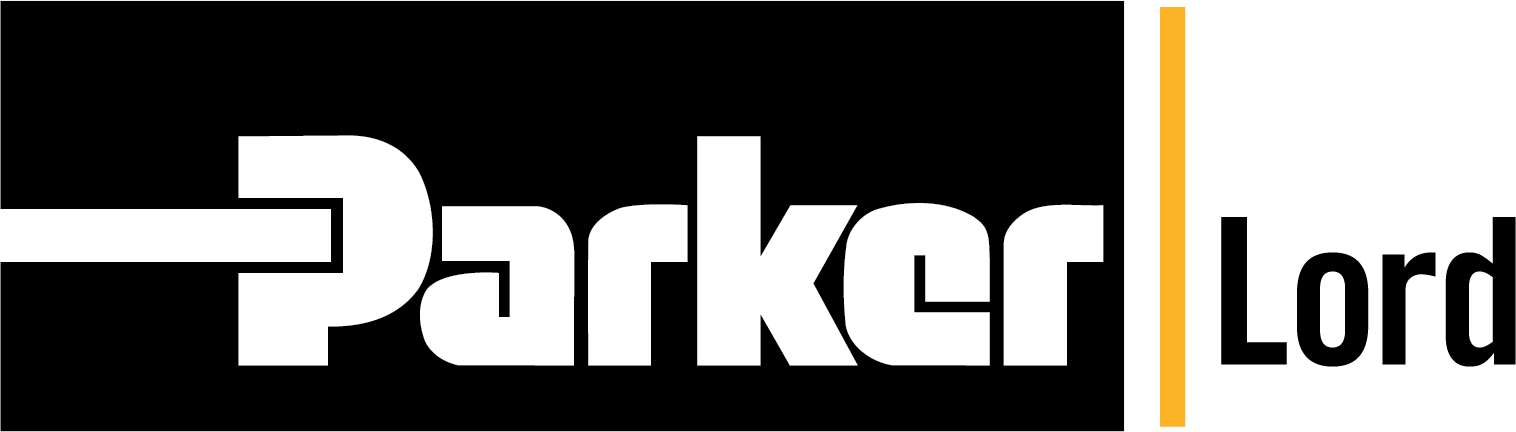
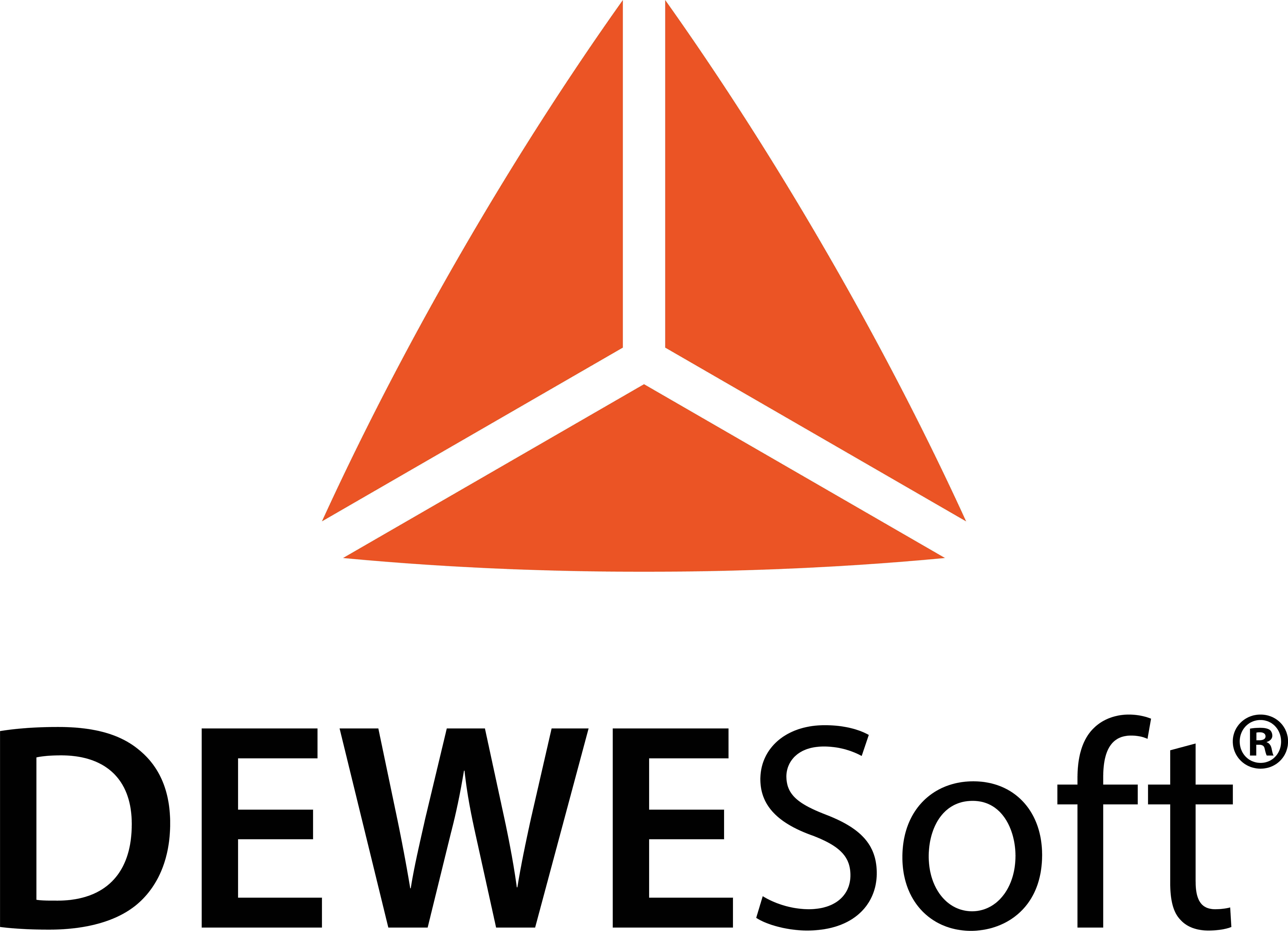
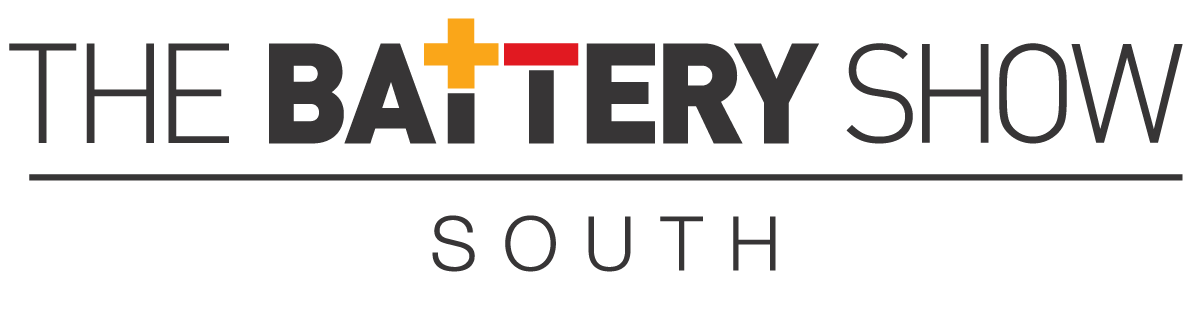
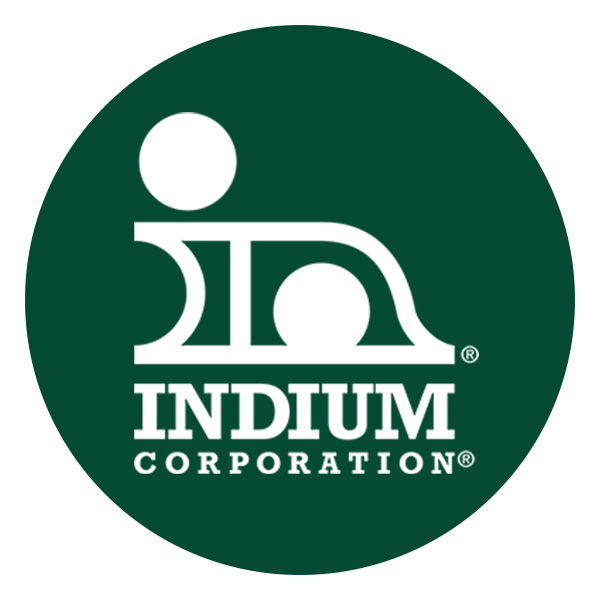
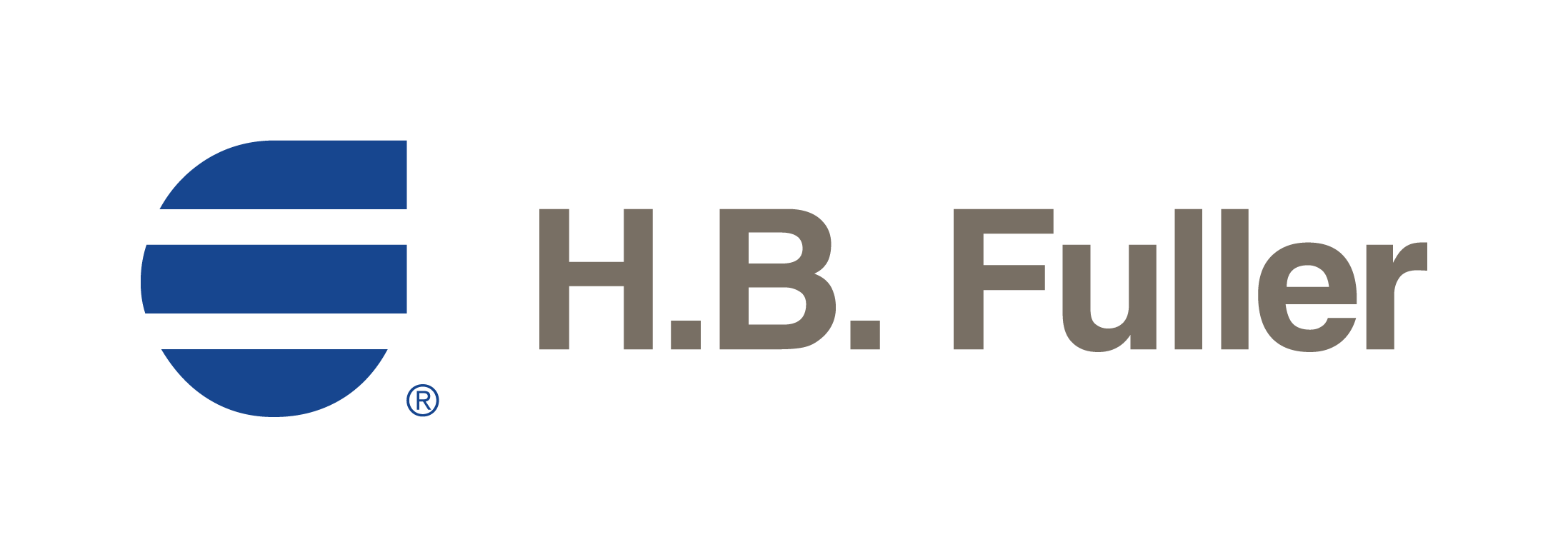